Introduzione
The artificial intelligence revolution has created unprecedented thermal management challenges, fundamentally transforming the economics of data center cooling. As organizations deploy increasingly powerful GPUs and specialized AI accelerators to train and run complex models, the financial implications of cooling technology selection extend far beyond initial capital costs. This comprehensive article explores the economic considerations of AI server cooling, providing a framework for cost-benefit analysis and strategies for maximizing return on investment.
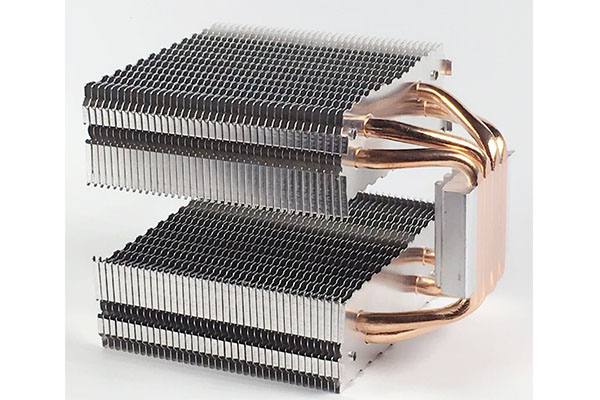
Sommario
- The Economic Impact of AI Cooling Decisions
- Capital Expenditure Analysis
- Operational Cost Considerations
- Performance Economics
- Density and Space Economics
- Total Cost of Ownership Framework
- ROI Optimization Strategies
- Domande frequenti
The Economic Impact of AI Cooling Decisions
Cooling technology selection has profound economic implications that extend throughout the AI infrastructure lifecycle.
Problem: Organizations often focus primarily on initial capital costs when evaluating cooling technologies, missing the broader economic impact.
The true economic impact of cooling technology selection includes operational costs, performance implications, reliability effects, and scaling considerations that are frequently undervalued in decision-making.
Aggravation: The economic equation for cooling is becoming increasingly complex as AI hardware costs, energy prices, and performance requirements evolve.
Further complicating matters, the rapid evolution of AI capabilities and hardware creates a dynamic economic landscape where the optimal cooling approach may change significantly over a system’s lifetime.
Soluzione: A comprehensive economic analysis that considers all cost and value factors enables more informed cooling technology decisions:
The Evolving Economics of AI Infrastructure
Understanding the financial context of cooling decisions:
- AI Hardware Investment Trends:
- High-end AI GPUs: $10,000-40,000 per device
- Multi-GPU servers: $50,000-500,000 per system
- AI clusters: $1-100+ million total investment
- Accelerating refresh cycles (2-3 years typical)
- Growing percentage of total IT spend
- Cooling as a Strategic Investment:
- Shift from operational expense to strategic enabler
- Impact on computational capacity and capability
- Influence on infrastructure deployment timelines
- Effect on competitive positioning and capabilities
- Relationship to overall AI strategy and outcomes
- Economic Evaluation Evolution:
- Traditional focus on initial capital costs
- Emerging emphasis on total cost of ownership
- Growing recognition of performance economics
- Increasing consideration of opportunity costs
- Development of comprehensive ROI frameworks
Here’s what makes this fascinating: The financial impact of cooling decisions has grown dramatically with the increasing value of AI computation. For traditional IT workloads, cooling typically represented 2-5% of total infrastructure costs and had minimal impact on computational output. For modern AI infrastructure, cooling can represent 8-15% of total costs and can impact computational output by 10-30% through its effect on performance, reliability, and density. This “cooling impact multiplier” has transformed cooling from a necessary but low-value expense to a strategic investment with significant ROI potential.
The Cost of Inadequate Cooling
Quantifying the financial impact of cooling limitations:
- Performance Degradation Costs:
- Thermal throttling reducing computational capacity by 10-30%
- Extended training times increasing time-to-results
- Inconsistent inference performance affecting service quality
- Reduced hardware utilization efficiency
- Opportunity costs of delayed AI implementation
- Reliability and Availability Impact:
- Increased failure rates from elevated temperatures
- Higher maintenance and replacement costs
- Downtime and service interruption expenses
- Reduced hardware lifespan and accelerated depreciation
- Support and troubleshooting resource requirements
- Scaling and Growth Limitations:
- Density constraints limiting computational capacity
- Facility limitations restricting expansion
- Delayed capability deployment due to infrastructure constraints
- Competitive disadvantage from capability limitations
- Strategic opportunity costs
But here’s an interesting phenomenon: The cost of inadequate cooling increases non-linearly with scale and density. For small AI deployments (10-50 GPUs), inadequate cooling might reduce effective capacity by 10-15% and increase operational costs by a similar amount. For large deployments (500+ GPUs), these impacts typically grow to 20-40% capacity reduction and 30-50% higher operational costs due to compound effects across the infrastructure. This “scale penalty” means that cooling becomes increasingly critical as AI deployments grow, fundamentally changing the ROI equation for advanced cooling technologies.
Economic Decision Framework
Developing a structured approach to cooling investment decisions:
- Comprehensive Cost Identification:
- Direct capital expenditures
- Implementation and transition costs
- Operational expenses over system lifetime
- Performance and capability implications
- Risk and reliability considerations
- Value and Benefit Quantification:
- Computational capacity improvements
- Energy and operational savings
- Space and infrastructure efficiency
- Hardware lifespan extension
- Strategic capability enablement
- Decision Criteria Development:
- Return on investment thresholds
- Payback period requirements
- Strategic alignment considerations
- Risk tolerance and mitigation
- Organizational priorities and constraints
| Economic Impact of Cooling Technology Selection |
|————————————————|
Fattore | Traditional Cooling | Advanced Cooling | Economic Differential | Calculation Approach |
---|---|---|---|---|
Capital Cost | $ | $$-$$$ | 2-3x higher initial investment | Direct comparison of implementation costs |
Energy Cost | $$$ | $ | 30-60% lower operational cost | kWh pricing × efficiency difference |
Density Impact | Baseline | 2-5x higher | 50-80% space cost reduction | Space cost × density improvement |
Performance Impact | -10 to -30% | Baseline | 10-30% effective capacity increase | Hardware cost × performance improvement |
Hardware Lifespan | Baseline | +20 to +40% | 20-40% replacement cost reduction | Replacement frequency × hardware cost |
Maintenance Cost | $ | $$-$$$ | 30-50% higher maintenance | Direct comparison of service costs |
3-Year TCO (Small) | Baseline | 0 to +20% | Potentially higher total cost | Comprehensive calculation of all factors |
3-Year TCO (Large) | Baseline | -20 to -40% | Significantly lower total cost | Comprehensive calculation of all factors |
Ready for the fascinating part? The most sophisticated organizations are implementing “cooling portfolio strategies” rather than standardizing on a single approach. By deploying different cooling technologies for different workloads and deployment scenarios, these organizations optimize both performance and economics across their AI infrastructure. Some have found that a carefully balanced portfolio approach can improve overall price-performance by 20-40% compared to homogeneous deployments, while simultaneously providing greater flexibility to adapt to evolving requirements. This portfolio approach represents a fundamental shift from viewing cooling as a standardized infrastructure component to treating it as a strategic resource that should be optimized for specific use cases.
Capital Expenditure Analysis
Understanding the initial investment requirements for different cooling approaches is essential for effective decision-making.
Problem: The significant capital cost differential between cooling technologies creates challenging investment decisions, particularly for budget-constrained organizations.
The 2-5x higher initial cost of advanced cooling technologies compared to traditional approaches creates substantial budget pressure and often becomes a primary obstacle to adoption despite potential long-term benefits.
Aggravation: The capital cost equation is complicated by facility modification requirements that vary significantly based on existing infrastructure.
Further complicating matters, many organizations struggle to accurately forecast the full implementation costs of advanced cooling, leading to budget surprises and project challenges.
Soluzione: A comprehensive capital expenditure analysis enables more accurate budgeting and investment planning:
Direct Equipment Costs
Understanding the hardware investment requirements:
- Cooling Technology Cost Comparison:
- Traditional air cooling: $200-500 per kW
- Advanced air cooling: $500-1,000 per kW
- Direct liquid cooling: $1,000-2,500 per kW
- Immersion cooling: $2,000-5,000 per kW
- Emerging technologies: $1,500-3,500 per kW
- Component-Level Cost Breakdown:
- Heat rejection equipment (chillers, cooling towers)
- Distribution infrastructure (piping, manifolds)
- Server-level cooling components (cold plates, heat exchangers)
- Control and monitoring systems
- Redundancy and backup systems
- Scale Economics Considerations:
- Volume discount opportunities
- Standardization benefits
- Deployment efficiency improvements
- Vendor partnership advantages
- Long-term agreement considerations
Here’s what makes this fascinating: The capital cost premium of advanced cooling technologies decreases significantly with scale. For small deployments (under 100 GPUs), advanced cooling might carry a 3-4x cost premium over air cooling. For large deployments (1000+ GPUs), economies of scale typically reduce this premium to 1.5-2x. This “scale effect” creates a compelling case for larger organizations to adopt advanced cooling, while smaller deployments may find the economics more challenging unless other factors like space constraints or performance requirements are significant drivers.
Implementation and Transition Costs
Accounting for the full deployment investment:
- Facility Modification Requirements:
- Structural reinforcement for weight
- Electrical system upgrades
- Plumbing and water distribution
- Heat rejection capacity expansion
- Space reconfiguration and preparation
- Installation and Commissioning Expenses:
- Engineering and design services
- Installation labor and materials
- Testing and validation
- Documentation and training
- Project management and oversight
- Transition and Migration Considerations:
- Phased implementation planning
- Temporary infrastructure requirements
- Operational continuity during transition
- Hardware migration costs
- Parallel operation expenses
But here’s an interesting phenomenon: The implementation cost differential between retrofitting existing facilities and new construction is creating a significant shift in deployment strategies. Retrofitting existing facilities for advanced cooling typically adds 30-60% to base technology costs, while incorporating advanced cooling into new construction might add only 10-20%. This “retrofit premium” is driving many organizations to deploy new AI infrastructure in purpose-built facilities rather than attempting to adapt existing data centers, fundamentally changing facility strategy for high-density AI deployments.
Financing and Investment Approaches
Optimizing the capital structure for cooling investments:
- Capital Allocation Strategies:
- CapEx vs. OpEx considerations
- Budget cycle alignment
- Multi-year investment planning
- Phased deployment approaches
- Reserve allocation for future expansion
- Alternative Financing Models:
- Cooling-as-a-Service options
- Vendor financing programs
- Leasing and subscription models
- Performance-based contracting
- Shared risk/reward structures
- Investment Justification Frameworks:
- Business case development
- ROI and payback calculation
- Strategic value articulation
- Risk mitigation quantification
- Competitive advantage demonstration
| Capital Cost Comparison by Cooling Technology and Scale |
|——————————————————–|
Tecnologia | Small Deployment (50 GPUs) | Medium Deployment (200 GPUs) | Large Deployment (1000+ GPUs) | Cost Drivers | Economies of Scale |
---|---|---|---|---|---|
Traditional Air | $50-100K | $150-300K | $500K-1M | Fans, heat sinks, airflow management | Minimal (10-20%) |
Advanced Air | $100-200K | $300-500K | $1-2M | Precision cooling, containment | Moderate (20-30%) |
Direct Liquid | $200-400K | $500K-1M | $2-4M | Cold plates, CDUs, distribution | Significant (30-50%) |
Immersion | $400-800K | $1-2M | $3-6M | Tanks, fluid, heat exchangers | Very High (40-60%) |
Hybrid Approach | $150-300K | $400-800K | $1.5-3M | Mixed technology implementation | Moderate (25-40%) |
Capital Efficiency Optimization
Maximizing the return on cooling capital investment:
- Modular and Scalable Deployment:
- Incremental capacity addition
- Just-in-time investment approach
- Standardized building blocks
- Future expansion accommodation
- Technology insertion planning
- Reuse and Repurposing Strategies:
- Existing infrastructure adaptation
- Component reuse opportunities
- Phased technology transition
- Legacy equipment integration
- Hybrid approach implementation
- Value Engineering Approaches:
- Design optimization for cost efficiency
- Specification right-sizing
- Vendor and solution comparison
- Competitive procurement strategies
- Implementation efficiency improvement
Ready for the fascinating part? The most sophisticated organizations are implementing “just-in-time” cooling infrastructure strategies that deploy capacity in smaller, more frequent increments rather than large upfront investments. By adopting modular, standardized cooling building blocks that can be deployed quickly as needed, these organizations are reducing initial capital requirements by 30-50% while maintaining the ability to scale rapidly. This approach not only improves capital efficiency but also reduces the risk of overbuilding or underbuilding capacity, creating a more agile infrastructure that can adapt to changing AI requirements.
Operational Cost Considerations
The ongoing operational expenses associated with cooling technology can significantly impact total cost of ownership and long-term economics.
Problem: Organizations often underestimate the operational cost differential between cooling technologies, focusing primarily on initial capital expenses.
The substantial differences in energy consumption, maintenance requirements, and reliability impacts between cooling technologies create significant operational cost variations that must be considered in economic analysis.
Aggravation: Operational costs are highly dependent on local factors like energy prices, climate conditions, and labor costs, creating complex regional variations.
Further complicating matters, the operational cost equation is evolving as energy prices increase, sustainability requirements grow, and the value of computational capacity rises.
Soluzione: A comprehensive operational cost analysis enables more accurate total cost of ownership calculation and better long-term decision-making:
Energy Consumption Analysis
Understanding the largest operational cost component:
- Cooling Energy Efficiency Comparison:
- Traditional air cooling: PUE 1.6-2.0 typical
- Advanced air cooling: PUE 1.4-1.7 typical
- Direct liquid cooling: PUE 1.1-1.3 typical
- Immersion cooling: PUE 1.03-1.15 typical
- Energy cost impact over system lifetime
- Efficiency Drivers and Considerations:
- Fan power requirements for air cooling
- Pump energy for liquid distribution
- Temperature differential advantages
- Free cooling opportunity expansion
- Heat reuse potential
- Location-Specific Energy Factors:
- Electricity cost variation ($0.05-0.30/kWh)
- Climate impact on free cooling potential
- Renewable energy availability
- Demand charges and time-of-use pricing
- Carbon taxation and regulatory factors
Here’s what makes this fascinating: The operational cost differential between cooling technologies varies dramatically based on energy costs and utilization patterns. In regions with low electricity costs ($0.05-0.08/kWh), the operational savings of advanced cooling might take 3-5 years to offset the higher capital costs. In high-cost energy regions ($0.20-0.30/kWh), this payback period can shrink to 1-2 years, fundamentally changing the economic equation. This “energy cost multiplier” means that optimal cooling selection should vary significantly based on deployment location and local energy economics.
Maintenance and Support Costs
Evaluating ongoing service requirements:
- Maintenance Requirement Comparison:
- Traditional air cooling: Lower complexity, higher frequency
- Direct liquid cooling: Moderate complexity, moderate frequency
- Immersion cooling: Higher complexity, lower frequency
- Preventative maintenance program differences
- Specialized expertise requirements
- Consumable and Replacement Considerations:
- Filter replacement for air cooling
- Fluid maintenance for liquid systems
- Component replacement frequencies
- Spare parts inventory requirements
- Consumable quality and longevity factors
- Support Model and Resource Requirements:
- In-house vs. contracted maintenance
- Staff training and certification needs
- Vendor support agreement options
- Remote monitoring capabilities
- Emergency response considerations
But here’s an interesting phenomenon: The maintenance requirements for advanced cooling technologies often follow a distinct “bathtub curve” that differs from traditional perceptions. While conventional wisdom suggests liquid cooling introduces maintenance complexity, data from mature implementations shows that after an initial break-in period with higher maintenance requirements, properly designed liquid cooling systems often require less frequent intervention than air-cooled systems. This maintenance profile creates a situation where initial operational costs may be higher but decrease over time as systems stabilize and staff expertise develops.
Reliability and Availability Impact
Quantifying the economic effect of cooling on system reliability:
- Failure Rate and Downtime Considerations:
- Temperature impact on component failure rates
- Cooling system reliability differences
- Mean time between failures comparison
- Mean time to repair variation
- Downtime cost implications
- Hardware Lifespan Extension Value:
- Temperature reduction effect on longevity
- Thermal cycling reduction benefits
- Humidity and contamination control
- Component replacement frequency
- Depreciation and amortization implications
- Business Continuity Considerations:
- Critical workload protection
- Redundancy and failover capabilities
- Graceful degradation options
- Recovery time objectives
- Business impact minimization
| Operational Cost Comparison by Cooling Technology |
Cost Category | Traditional Air | Advanced Air | Direct Liquid | Immersion | Key Variables |
---|---|---|---|---|---|
Energy (PUE) | 1.6-2.0 | 1.4-1.7 | 1.1-1.3 | 1.03-1.15 | Local electricity cost, utilization |
Maintenance | $$ | $$ | $$$ | $$$$ | Staff expertise, vendor support |
Consumables | $ | $ | $$ | $$$ | Quality, replacement frequency |
Reliability Impact | $$$ | $$ | $ | $ | Workload value, redundancy |
Hardware Lifespan | Baseline | +10-20% | +20-40% | +30-50% | Hardware cost, refresh cycle |
Staff Resources | $ | $$ | $$$ | $$$$ | Labor cost, training investment |
Total OpEx (3yr) | $$$$$ | $$$$ | $$$ | $$ | Scale, location, implementation |
Staffing and Operational Overhead
Accounting for human resource requirements:
- Staffing Model Comparison:
- Traditional cooling: General IT skills
- Advanced cooling: Specialized expertise
- Staffing level requirements
- Training and certification investment
- Career development considerations
- Operational Procedure Differences:
- Monitoring and management activities
- Preventative maintenance procedures
- Troubleshooting and response protocols
- Documentation and compliance requirements
- Continuous improvement processes
- Organizational Impact Considerations:
- IT and facilities integration requirements
- Responsibility and ownership models
- Cross-functional collaboration needs
- Knowledge management approaches
- Organizational change management
Ready for the fascinating part? The most sophisticated organizations are implementing “cooling operations centers” that centralize expertise and monitoring across multiple facilities. By creating specialized teams with deep cooling technology knowledge, these organizations achieve 30-50% lower operational costs compared to distributed management models, while simultaneously improving reliability and performance. This centralized approach enables more efficient resource allocation, better knowledge sharing, and more consistent operations across the infrastructure portfolio, fundamentally changing how cooling systems are managed and optimized.
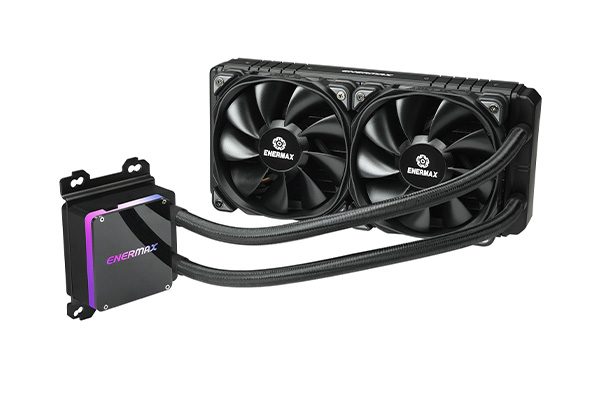
Performance Economics
The impact of cooling technology on computational performance creates significant economic implications that are often undervalued in decision-making.
Problem: Organizations frequently overlook the performance impact of cooling when evaluating technology options, missing a critical economic factor.
The potential 10-30% performance differential between adequate and inadequate cooling creates substantial economic implications through effective computational capacity, training time, and hardware utilization efficiency.
Aggravation: The performance impact of cooling varies significantly based on workload characteristics, hardware configuration, and utilization patterns.
Further complicating matters, the economic value of performance is highly dependent on specific use cases, making standardized valuation challenging and often leading to undervaluation in decision processes.
Soluzione: A comprehensive performance economics analysis enables more accurate valuation of cooling technology benefits:
Thermal Throttling Prevention
Quantifying the value of maintaining full computational capacity:
- Performance Loss Quantification:
- GPU throttling behavior under thermal stress
- Clock speed reduction percentages
- Memory bandwidth impact
- Computational throughput reduction
- Workload completion time extension
- Workload-Specific Impact Analysis:
- Training workload completion time effects
- Inference throughput and latency implications
- Batch size optimization constraints
- Model complexity limitations
- Development and research velocity impact
- Economic Value Calculation:
- Hardware utilization efficiency improvement
- Effective cost per computation reduction
- Time-to-result acceleration value
- Additional workload capacity enablement
- Competitive advantage quantification
Here’s what makes this fascinating: Research indicates that inadequate cooling can reduce the effective computational capacity of AI infrastructure by 15-40%, essentially negating much of the performance advantage of premium GPU hardware. This “thermal tax” means that organizations may be realizing only 60-85% of their theoretical computing capacity due to cooling limitations, fundamentally changing the economics of AI infrastructure. When combined with the reliability impact, the total cost of inadequate cooling can exceed the price premium of advanced cooling solutions within the first year of operation for high-utilization AI systems.
Hardware Utilization Optimization
Maximizing return on AI hardware investment:
- Capital Utilization Improvement:
- Hardware investment utilization percentage
- Effective cost per training run
- Computational capacity per dollar invested
- Asset utilization metrics
- Return on hardware investment
- Workload Throughput Enhancement:
- Training iterations per day increase
- Inference queries per second improvement
- Batch processing capacity expansion
- Development cycle acceleration
- Research capability enhancement
- Scaling Efficiency Considerations:
- Multi-GPU scaling effectiveness
- Cluster-wide performance consistency
- Distributed training efficiency
- Resource allocation optimization
- Infrastructure scaling economics
But here’s an interesting phenomenon: The performance impact of cooling increases non-linearly with scale and density. For single-GPU workstations, the performance differential between cooling technologies might be 5-10%. For 8-GPU servers, this typically grows to 10-20%, and for large clusters with hundreds of GPUs, the impact can reach 20-30% due to compound effects of thermal limitations across the infrastructure. This “scale multiplier” means that the performance economics of advanced cooling become increasingly compelling as deployments grow, creating a strong correlation between scale and cooling technology sophistication.
Business Value Considerations
Connecting cooling performance to organizational outcomes:
- Time-to-Market Advantages:
- AI model development acceleration
- Product release timeline improvement
- Competitive response capability
- Market opportunity capture
- First-mover advantage enablement
- Research and Innovation Enablement:
- Experimental iteration increase
- Model complexity expansion capability
- Research scope broadening
- Innovation cycle acceleration
- Breakthrough potential enhancement
- Service Quality and Reliability:
- Inference response time consistency
- Service level agreement compliance
- User experience improvement
- System stability enhancement
- Reputation and trust building
| Performance Impact of Cooling Technology Selection |
Workload Type | Performance Impact | Economic Value Drivers | Cooling Technology Benefit | ROI Calculation Approach |
---|---|---|---|---|
AI Training | 10-30% faster completion | Time-to-result, researcher productivity | Consistent maximum performance | Training cost × time reduction |
Inference Services | 5-20% higher throughput | Service capacity, response time | Stable performance, higher density | Revenue per query × capacity increase |
Research & Development | 15-25% more experiments | Innovation velocity, competitive advantage | Reliable operation, maximum capability | R&D cost × productivity improvement |
Batch Processing | 10-20% higher throughput | Processing capacity, time-to-insight | Sustained performance for long jobs | Processing cost × throughput increase |
Mixed Workloads | 10-15% overall improvement | Resource utilization, flexibility | Adaptability to varying thermal loads | Blended calculation based on workload mix |
Performance Consistency Value
Evaluating the economic impact of stable computational capacity:
- Thermal Stability Benefits:
- Performance consistency over time
- Predictable completion timelines
- Reliable resource scheduling
- Consistent user experience
- Reproducible research results
- Workload Planning Improvement:
- Accurate completion time estimation
- Reliable resource allocation
- Predictable capacity planning
- Consistent service level delivery
- Operational predictability enhancement
- Quality of Service Considerations:
- Consistent inference latency
- Reliable batch processing windows
- Stable interactive performance
- Predictable system behavior
- User satisfaction improvement
Ready for the fascinating part? The most sophisticated organizations are implementing “performance-based cooling” strategies that dynamically allocate cooling resources based on workload value and performance sensitivity. By creating tiered cooling service levels aligned with workload requirements, these organizations optimize both cooling investment and computational performance across their infrastructure. Some have found that this value-based approach can improve overall price-performance by 15-30% compared to uniform cooling implementations, while simultaneously providing greater flexibility to adapt to evolving requirements. This approach represents a fundamental shift from viewing cooling as infrastructure to treating it as a service that should be aligned with specific workload requirements and business value.
Density and Space Economics
The impact of cooling technology on deployment density creates significant economic implications through space utilization, infrastructure efficiency, and scaling capabilities.
Problem: Traditional cooling approaches create density limitations that constrain AI infrastructure deployment and scaling.
The practical density limits of air cooling (typically 15-25kW per rack) create significant space requirements for large AI deployments, driving up real estate costs and limiting deployment options.
Aggravation: Data center space costs vary dramatically by location, creating complex regional economics for density considerations.
Further complicating matters, many organizations face absolute space constraints in existing facilities, creating situations where density becomes a primary decision driver regardless of other economic factors.
Soluzione: A comprehensive analysis of density economics enables more effective facility planning and cooling technology selection:
Space Utilization Optimization
Quantifying the economic value of density:
- Data Center Space Cost Analysis:
- Facility construction costs ($1,000-3,000 per square foot)
- Real estate expenses by location
- Operational costs per square foot
- Expansion and growth accommodation
- Total facility lifecycle economics
- Density Comparison by Cooling Technology:
- Traditional air cooling: 4-8 kW per rack typical
- Advanced air cooling: 15-25 kW per rack
- Direct liquid cooling: 30-80 kW per rack
- Immersion cooling: 50-150 kW per rack
- Space requirement differential calculation
- Computational Density Metrics:
- GPUs per rack comparison
- Compute capacity per square foot
- Performance per square foot
- Space efficiency improvement percentage
- Total capacity within space constraints
Here’s what makes this fascinating: The density advantage of advanced cooling creates dramatic space efficiency improvements that fundamentally change data center economics. While traditional air-cooled data centers might support 5-10kW per rack and require 8-10 square feet per kW of IT load, advanced cooling can support 50-100kW per rack and require just 1-2 square feet per kW. This 5-8x improvement in spatial efficiency can reduce data center construction costs by 40-60% per unit of computing capacity, creating compelling economics despite the higher cost of the cooling technology itself. For organizations in space-constrained environments or high-cost real estate markets, this density advantage can be the primary driver for advanced cooling adoption, even before considering the performance and efficiency benefits.
Infrastructure Efficiency Improvement
Leveraging density for broader infrastructure optimization:
- Power Distribution Efficiency:
- Cable and busway length reduction
- Power distribution losses minimization
- Transformer and UPS sizing optimization
- Electrical infrastructure cost reduction
- Power system reliability improvement
- Networking Infrastructure Benefits:
- Cable length and complexity reduction
- Switch and router consolidation
- Latency minimization through proximity
- Network infrastructure cost savings
- Simplified topology and management
- Facility System Optimization:
- Mechanical distribution efficiency
- Building management simplification
- Security and access control consolidation
- Fire protection system optimization
- Overall infrastructure cost reduction
But here’s an interesting phenomenon: The infrastructure efficiency benefits of density follow a non-linear curve with increasing returns as density grows. Doubling density typically reduces infrastructure costs by more than 50% due to the compound effect across multiple systems. This “density multiplier” creates a situation where the infrastructure savings from advanced cooling can often exceed the cooling technology premium itself, particularly for new construction where all infrastructure components can be optimized for the higher density from the beginning.
Scaling and Growth Accommodation
Enabling future expansion through density optimization:
- Facility Capacity Maximization:
- Total computational capacity within existing space
- Growth accommodation without expansion
- Phased deployment within fixed footprint
- Infrastructure utilization optimization
- Capital investment deferral through density
- Expansion Strategy Optimization:
- Incremental growth capability
- Reduced future construction requirements
- Faster capacity addition timelines
- Lower expansion capital requirements
- Strategic flexibility enhancement
- Location Strategy Considerations:
- High-cost location viability improvement
- Proximity to business operations enablement
- Edge deployment density requirements
- Geographic distribution optimization
- Location constraint mitigation
| Density and Space Economics by Cooling Technology |
|————————————————–|
Metric | Traditional Air | Advanced Air | Direct Liquid | Immersion | Economic Impact |
---|---|---|---|---|---|
Rack Density | 5-15 kW | 15-25 kW | 30-80 kW | 50-150 kW | Space requirement, infrastructure efficiency |
GPUs per Rack | 8-16 | 16-32 | 32-64 | 48-96+ | Computational density, management efficiency |
Space per kW | 8-10 sq ft | 4-6 sq ft | 2-3 sq ft | 1-2 sq ft | Facility size, construction cost |
Power Density | 150-300 W/sq ft | 300-600 W/sq ft | 600-1200 W/sq ft | 900-1800 W/sq ft | Infrastructure utilization, expansion needs |
Scaling Increment | Large (1-2MW) | Moderate (500kW-1MW) | Small (100-500kW) | Very Small (50-200kW) | Deployment flexibility, capital efficiency |
Facility Lifecycle Considerations
Evaluating long-term facility economics:
- Initial Construction Optimization:
- Facility size reduction through density
- Infrastructure right-sizing opportunities
- Construction timeline acceleration
- Capital investment optimization
- Faster time-to-deployment capability
- Operational Lifecycle Benefits:
- Reduced ongoing facility expenses
- Simplified management and maintenance
- Lower total energy consumption
- Improved space utilization over time
- Extended useful facility lifetime
- Repurposing and Adaptation Flexibility:
- Technology transition accommodation
- Workload evolution support
- Infrastructure modernization simplification
- Future cooling technology adoption
- Long-term facility strategy optimization
Ready for the fascinating part? The most advanced organizations are implementing “density-first” facility strategies that prioritize computational density above most other factors. By designing facilities specifically for maximum density through advanced cooling, these organizations are achieving 3-5x higher computational capacity per square foot compared to traditional designs, with 30-50% lower construction costs per unit of computing capacity. This approach represents one of the most significant shifts in data center design since the introduction of raised floors, driven primarily by the unique requirements of AI infrastructure and the economic value of computational density.
Total Cost of Ownership Framework
A comprehensive total cost of ownership (TCO) analysis is essential for making informed cooling technology decisions that optimize long-term economics.
Problem: The complex interplay of capital, operational, performance, and density factors creates challenging economic analysis requirements.
The diverse cost and value components of cooling technology selection, combined with their varying timelines and dependencies, make simplified comparison approaches inadequate for effective decision-making.
Aggravation: The rapid evolution of both AI hardware and cooling technology creates a dynamic economic landscape that complicates long-term analysis.
Further complicating matters, many organizations lack comprehensive data on the full economic impact of cooling technologies, creating uncertainty in TCO calculations and potentially leading to suboptimal decisions.
Soluzione: A structured TCO framework enables more accurate economic analysis and better-informed cooling technology decisions:
Comprehensive Cost Component Identification
Ensuring all relevant factors are included:
- Direct Cost Categories:
- Initial capital expenditure
- Installation and commissioning costs
- Energy expenses over system lifetime
- Maintenance and support costs
- Staffing and operational expenses
- Consumables and replacement parts
- End-of-life decommissioning expenses
- Indirect Cost Considerations:
- Space and real estate expenses
- Infrastructure overhead allocation
- Downtime and reliability impact
- Performance and capacity effects
- Scaling and growth implications
- Risk and compliance factors
- Timeline and Lifecycle Factors:
- Analysis period definition (typically 3-5 years)
- Technology refresh considerations
- Expansion and growth projections
- Infrastructure lifecycle alignment
- Time value of money calculations
Here’s what makes this fascinating: The most effective TCO analyses are implementing “value-adjusted TCO” approaches that incorporate both cost components and value factors into a unified framework. By quantifying the economic impact of performance, reliability, and scaling benefits alongside traditional cost elements, these analyses provide a more complete picture of the true economic impact of cooling decisions. Organizations using this approach report making significantly different technology selections compared to traditional cost-focused analysis, typically favoring more advanced cooling technologies due to their broader value contribution despite higher initial costs.
Scenario-Based Analysis Methodology
Addressing uncertainty and variability:
- Baseline Scenario Development:
- Current requirements and constraints
- Expected growth and evolution
- Typical utilization patterns
- Standard economic assumptions
- Probable technology progression
- Alternative Scenario Exploration:
- Accelerated growth possibilities
- Delayed or reduced expansion
- Technology evolution variations
- Economic condition changes
- Workload characteristic shifts
- Sensitivity Analysis Implementation:
- Energy price variation impact
- Utilization level effects
- Capital cost fluctuation influence
- Performance value sensitivity
- Space cost and constraint changes
But here’s an interesting phenomenon: The TCO advantage of advanced cooling technologies increases non-linearly with scale and density. For small deployments (under 100 GPUs), advanced cooling might carry a 10-20% TCO premium over traditional approaches. For large deployments (1000+ GPUs), advanced cooling typically delivers a 20-40% TCO advantage due to density benefits, efficiency improvements, and performance gains. This “scale effect” means that the economic equation for cooling technology selection should vary significantly based on deployment size, with larger deployments more easily justifying advanced approaches.
Comparative Analysis Framework
Enabling effective technology comparison:
- Technology Option Definition:
- Traditional air cooling baseline
- Advanced air cooling alternatives
- Direct liquid cooling options
- Immersion cooling approaches
- Hybrid and emerging technologies
- Standardized Comparison Methodology:
- Consistent assumption application
- Equivalent analysis periods
- Normalized performance metrics
- Comparable reliability factors
- Aligned growth projections
- Decision Support Visualization:
- Total cost comparison charts
- Cost component breakdown analysis
- Cumulative cost curves over time
- Payback period calculation
- Return on investment visualization
| 5-Year TCO Comparison for 1MW AI Deployment |
Cost Component | Traditional Air | Advanced Air | Direct Liquid | Immersion | Cost Calculation Basis |
---|---|---|---|---|---|
Initial Capital | $2-3M | $3-4M | $5-7M | $8-12M | Equipment, installation, facility modifications |
Energy (5yr) | $8-12M | $6-9M | $4-6M | $3-5M | PUE × IT load × electricity cost × time |
Maintenance (5yr) | $1-2M | $1.5-2.5M | $2-3M | $2.5-3.5M | Annual service cost × time |
Space Cost (5yr) | $3-5M | $2-3M | $1-1.5M | $0.8-1.2M | Square footage × facility cost × time |
Performance Impact | -$3-5M | -$1-2M | $0 | +$1-2M | Effective capacity difference × workload value |
Hardware Lifespan | $0 | +$1-2M | +$2-3M | +$3-4M | Replacement frequency reduction × hardware cost |
Total 5-Year TCO | $17-27M | $13-19M | $14-20M | $15-24M | Sum of all components with adjustments |
TCO per Compute Unit | Baseline | 20-30% lower | 15-25% lower | 10-20% lower | TCO ÷ effective computational capacity |
Strategic Value Assessment
Incorporating broader business impact:
- Competitive Advantage Considerations:
- Time-to-market acceleration value
- Capability and capacity differentiation
- Innovation and research velocity
- Scaling and growth enablement
- Strategic flexibility enhancement
- Risk Mitigation Valuation:
- Reliability and availability improvement
- Business continuity enhancement
- Regulatory compliance assurance
- Future-proofing benefit
- Technology obsolescence protection
- Organizational Capability Development:
- Knowledge and expertise building
- Operational excellence advancement
- Vendor relationship development
- Technology leadership positioning
- Strategic partnership opportunities
Ready for the fascinating part? The most sophisticated organizations are implementing “cooling portfolio strategies” rather than standardizing on a single approach. By deploying different cooling technologies for different workloads and deployment scenarios, these organizations optimize both performance and economics across their AI infrastructure. Some have found that a carefully balanced portfolio approach can improve overall price-performance by 20-40% compared to homogeneous deployments, while simultaneously providing greater flexibility to adapt to evolving requirements. This portfolio approach represents a fundamental shift from viewing cooling as a standardized infrastructure component to treating it as a strategic resource that should be optimized for specific use cases.
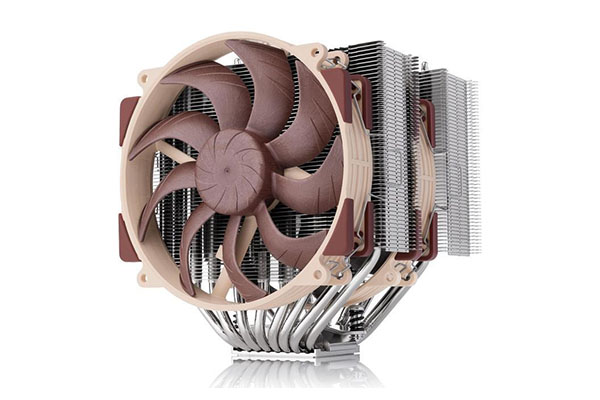
ROI Optimization Strategies
Maximizing the return on investment from cooling technology requires strategic approaches that go beyond simple technology selection.
Problem: Even with optimal technology selection, many organizations fail to realize the full potential value of their cooling investments.
Suboptimal implementation, operational practices, and ongoing management can significantly reduce the realized benefits of advanced cooling technologies, diminishing ROI despite appropriate technology selection.
Aggravation: The rapid evolution of AI workloads and hardware creates a dynamic environment that requires continuous optimization to maintain ROI.
Further complicating matters, many organizations lack the specialized expertise and processes needed to fully optimize cooling system performance and efficiency over time.
Soluzione: Implementing comprehensive ROI optimization strategies enables organizations to maximize the value of their cooling investments:
Implementation Optimization
Maximizing value from the deployment phase:
- Phased Deployment Strategies:
- Pilot and proof of concept implementation
- Targeted high-value application
- Incremental expansion approach
- Experience and expertise building
- Risk and disruption minimization
- Vendor Selection and Management:
- Comprehensive evaluation criteria
- Total value assessment beyond price
- Partnership approach development
- Knowledge transfer requirements
- Long-term relationship building
- Project Execution Excellence:
- Detailed planning and preparation
- Comprehensive testing and validation
- Thorough documentation development
- Effective knowledge transfer
- Operational readiness assurance
Here’s what makes this fascinating: Organizations that implement formal cooling technology pilot programs before full deployment typically achieve 15-25% better outcomes in terms of performance, reliability, and cost-effectiveness compared to those that move directly to production implementation. This “pilot advantage” stems from the opportunity to validate technology in the specific environment, develop internal expertise, refine operational procedures, and identify potential issues before large-scale deployment. The most successful organizations treat pilots as learning experiences rather than simply technology demonstrations, creating a foundation for successful scaling that significantly enhances overall ROI.
Operational Excellence Development
Ensuring ongoing optimization:
- Performance Monitoring and Optimization:
- Comprehensive measurement implementation
- Baseline establishment and tracking
- Regular performance analysis
- Continuous improvement processes
- Optimization opportunity identification
- Energy Efficiency Maximization:
- Operating parameter optimization
- Dynamic control implementation
- Seasonal adjustment strategies
- Free cooling maximization
- Heat reuse opportunity development
- Reliability and Availability Enhancement:
- Preventative maintenance optimization
- Predictive analytics implementation
- Component lifecycle management
- Failure mode analysis and mitigation
- Continuous reliability improvement
But here’s an interesting phenomenon: The operational practices for cooling systems have a larger impact on realized value than many organizations recognize. Research indicates that the difference between average and excellent operational practices can create a 20-30% variation in energy efficiency, reliability, and performance outcomes for identical cooling technologies. This “operational multiplier” means that investments in operational excellence can deliver ROI comparable to technology upgrades at a fraction of the cost, creating compelling economics for developing specialized expertise and optimized processes.
Value Capture Maximization
Ensuring benefits are fully realized:
- Performance Benefit Realization:
- Workload optimization for cooling capability
- GPU configuration adjustment
- Utilization pattern optimization
- Thermal monitoring integration
- Dynamic workload management
- Density Advantage Leveraging:
- Infrastructure consolidation
- Space repurposing and optimization
- Growth accommodation within existing facilities
- Expansion deferral through density
- Location strategy optimization
- Efficiency Benefit Monetization:
- Energy cost reduction capture
- Carbon footprint improvement quantification
- Sustainability goal advancement
- Regulatory compliance enhancement
- Corporate responsibility alignment
| ROI Optimization Strategies by Implementation Phase |
|—————————————————–|
Phase | Key Strategies | Potential Impact | Implementation Approach | Success Metrics |
---|---|---|---|---|
Planning | Comprehensive requirements analysis, technology alignment | 15-25% better outcomes | Structured assessment, stakeholder input | TCO reduction, alignment with needs |
Deployment | Phased implementation, knowledge transfer, testing | 20-30% fewer issues | Pilot programs, expertise development | Implementation time, budget adherence |
Early Operation | Baseline establishment, procedure development | 10-20% better initial performance | Measurement systems, documentation | Performance vs. expectations |
Ongoing Operation | Continuous optimization, predictive maintenance | 20-30% better long-term results | Monitoring, analysis, improvement cycles | Efficiency trends, reliability metrics |
Technology Transition | Planned upgrades, capability evolution | 25-40% lower transition costs | Roadmap development, modular approach | Upgrade costs, performance improvement |
Strategic Alignment Enhancement
Connecting cooling to broader organizational objectives:
- Business Objective Integration:
- AI strategy alignment
- Computational capability enablement
- Innovation and research acceleration
- Competitive differentiation support
- Strategic initiative enablement
- Sustainability Goal Advancement:
- Energy efficiency improvement
- Carbon footprint reduction
- Water conservation enhancement
- Waste heat utilization
- Environmental impact minimization
- Financial Performance Optimization:
- Capital efficiency improvement
- Operational cost reduction
- Asset utilization enhancement
- Investment timing optimization
- Total economic impact maximization
Ready for the fascinating part? The most sophisticated organizations are implementing “value-based cooling management” approaches that continuously align cooling resources with business priorities. By creating direct connections between cooling performance metrics and business outcomes, these organizations ensure that cooling optimization efforts focus on the highest-value opportunities rather than technical metrics alone. Some have found that this business-aligned approach can improve the perceived value of cooling investments by 2-3x compared to traditional technically-focused management, creating stronger support for ongoing investment and optimization. This approach represents a fundamental shift from viewing cooling as a technical function to treating it as a strategic business enabler that directly contributes to organizational success.
Domande frequenti
Q1: How do I build a comprehensive business case for advanced cooling technology investment?
Building a compelling business case for advanced cooling requires a comprehensive approach: First, quantify all cost components—beyond initial capital expenses, include energy costs, maintenance requirements, space utilization, and operational expenses over a 3-5 year horizon. Second, calculate performance economics—quantify the value of preventing thermal throttling (typically 10-30% of computational capacity), improving hardware utilization, and enabling consistent performance. For AI workloads, this performance impact often translates directly to faster time-to-results and higher throughput. Third, analyze density benefits—advanced cooling typically enables 3-5x higher density, reducing space requirements and associated infrastructure costs. In high-cost locations, these savings can exceed the cooling technology premium. Fourth, evaluate reliability improvements—lower operating temperatures typically extend hardware lifespan by 20-40% and reduce failure rates, creating significant value for expensive AI accelerators. Fifth, consider strategic benefits—faster deployment capability, greater scaling flexibility, and improved competitive positioning. The most effective business cases use scenario analysis to demonstrate outcomes under different growth, utilization, and economic assumptions, providing a robust foundation for decision-making. For large deployments (500+ GPUs), advanced cooling typically delivers 20-40% lower total cost of ownership despite higher initial investment, creating a compelling financial case independent of strategic benefits. For smaller deployments, the business case often hinges more on specific constraints like space limitations or performance requirements that may justify the investment despite potentially higher total costs.
Q2: What are the key economic differences between retrofitting existing facilities for advanced cooling versus building new purpose-built infrastructure?
The economic equation for retrofitting versus new construction presents several critical considerations: First, capital cost differential—retrofitting existing facilities for advanced cooling typically adds 30-60% to base technology costs, while incorporating advanced cooling into new construction might add only 10-20%. This “retrofit premium” stems from the challenges of adapting existing infrastructure, potential stranded investments, and implementation complexity. Second, operational impact—retrofits often require working around active systems, potentially creating disruption costs that new construction avoids. Third, performance optimization—purpose-built facilities can optimize all infrastructure elements for advanced cooling, potentially achieving 15-25% better efficiency and performance compared to retrofitted environments with their inherent compromises. Fourth, scaling economics—new construction can implement modular, standardized designs optimized for incremental growth, while retrofits often face constraints that limit future expansion or create inefficiencies. Fifth, timeline considerations—while retrofits leverage existing shell infrastructure, the complexity of working in operational environments often extends implementation timelines, potentially offsetting some of the time advantage. For organizations with substantial existing investment, the retrofit equation typically favors creating dedicated high-density zones within existing facilities rather than facility-wide conversion. This targeted approach optimizes investment for specific AI workloads while maintaining existing infrastructure for less demanding applications. For organizations planning significant AI growth, purpose-built facilities often deliver better long-term economics despite higher initial investment, particularly when considering the full lifecycle costs and performance benefits of optimized infrastructure.
Q3: How should cooling technology selection vary based on the scale and growth trajectory of AI infrastructure?
Cooling strategy should be tailored to both current scale and anticipated growth: For small-scale deployments (under 100 GPUs) with moderate growth expectations, simplicity and capital efficiency typically take priority. These environments often benefit most from standardizing on a single advanced cooling approach that balances performance and implementation complexity, such as direct-to-chip liquid cooling for GPUs with traditional cooling for other components. For medium-scale deployments (100-500 GPUs) with significant growth projections, flexibility and scalability become critical. These organizations typically benefit from modular approaches with clear technology transition points, potentially implementing a tiered strategy with different cooling technologies for different density requirements. For large-scale deployments (500+ GPUs) with rapid growth trajectories, long-term economics and maximum density typically drive decisions. These environments often justify comprehensive liquid cooling or immersion approaches that maximize performance and efficiency while enabling extreme density. Growth pattern also significantly impacts strategy: Linear, predictable growth favors building appropriate headroom into initial implementations, while unpredictable or exponential growth benefits from highly modular approaches that can scale incrementally. The most sophisticated organizations implement “cooling portfolio strategies” with different technologies for different workloads and deployment scenarios. This portfolio approach can improve overall price-performance by 20-40% compared to homogeneous deployments while providing greater flexibility to adapt to evolving requirements. The key is aligning cooling strategy with both current requirements and future growth patterns, recognizing that the optimal approach varies significantly based on scale, growth trajectory, and organizational priorities.
Q4: How does the economic equation for cooling technology vary based on geographic location and energy costs?
Geographic location creates significant variation in cooling economics through several key factors: First, energy cost impact—electricity prices ranging from $0.05-0.30/kWh create dramatic differences in operational expenses. In high-cost regions ($0.20+/kWh), the energy savings from advanced cooling can offset the higher capital costs within 1-2 years, while low-cost regions ($0.05-0.08/kWh) might require 3-5 years to reach payback. Second, climate considerations—locations with cool climates enable free cooling for more hours annually, potentially increasing the efficiency advantage of liquid cooling by enabling higher temperature operation. Third, real estate economics—in high-cost urban areas ($500-1000+ per square foot), the density advantage of advanced cooling creates substantial space-related savings that can exceed the technology premium. Fourth, water availability—regions with water scarcity may face restrictions or higher costs for water-based cooling, potentially favoring air-cooled or closed-loop approaches despite efficiency trade-offs. Fifth, regulatory environment—locations with strict energy efficiency requirements or carbon reduction mandates may create additional incentives for advanced cooling through compliance benefits or penalty avoidance. The most sophisticated organizations implement location-specific cooling strategies rather than global standardization, recognizing that the optimal approach varies significantly based on local conditions. For example, a deployment in Singapore (high energy costs, limited space, hot climate) might strongly favor immersion cooling despite the higher capital cost, while a deployment in Quebec (low energy costs, available space, cool climate) might find advanced air cooling more economically attractive. This geographic optimization can improve overall economics by 15-30% compared to standardized global approaches, creating compelling justification for location-specific strategies.
Q5: What are the most effective approaches for measuring and optimizing the ROI of cooling investments over time?
Maximizing cooling ROI requires comprehensive measurement and continuous optimization: First, implement multi-dimensional measurement—track not just direct cooling metrics (temperature, energy consumption) but also their impact on computational performance, hardware reliability, and operational efficiency. This holistic view provides a complete picture of realized value. Second, establish clear baselines—document pre-implementation performance, efficiency, and costs to enable accurate quantification of improvements. Third, develop comprehensive KPIs—create metrics that connect cooling performance to business outcomes, such as computational throughput per dollar, training time reduction, or effective capacity increase. Fourth, implement regular review cycles—conduct quarterly performance analysis to identify optimization opportunities, track benefit realization, and adjust operational parameters. Fifth, maintain technology awareness—continuously evaluate emerging cooling technologies and approaches against current implementation to identify potential upgrade opportunities and optimal transition timing. The most effective organizations implement “cooling value management” programs that treat cooling as a strategic asset rather than infrastructure. These programs typically include dedicated resources for optimization, regular executive reporting on value realization, and continuous improvement processes focused on maximizing return. Organizations with formal cooling optimization programs typically achieve 20-30% better ROI compared to passive management approaches, creating compelling economics for the additional focus and resources. The key success factor is maintaining a business-oriented perspective that connects cooling performance directly to organizational outcomes rather than treating it as a purely technical domain, ensuring that optimization efforts focus on the highest-value opportunities rather than technical metrics alone.